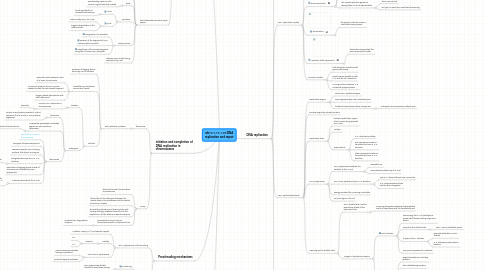
1. DNA repair
1.1. most spontaneous changes in DNA are temporary
1.1.1. corrected by a set of processes known as DNA repair mechanisms
1.2. evidence 4 importance of DNA repair
1.2.1. large investment that cells make in DNA repair enzymes
1.2.2. increased rate of mutation that happens when a DNA repair gene has been inactivated
1.2.3. many human diseases linked with decreased repair
1.2.3.1. e.g. XP
1.2.3.1.1. patients have an extreme sensitivity to UV light
1.2.3.1.2. unable to repair certain DNA photoproducts
1.3. without DNA repair
1.3.1. spontaneous changes=mutations if not repaired
1.3.1.1. sickle cell anemia
1.3.1.1.1. permanent change in single nucleotide in Hb gene
1.3.1.1.2. Hb with incorrect sequence of amino acids
1.3.2. DNA=highly stable but still susceptible to spontaneous changes
1.3.3. examples of spontaneous changes that could occur
1.3.3.1. depurination
1.3.3.1.1. DNA of human cell loses 5000 purine bases
1.3.3.1.2. N-glycosyl links with deoxyribose hydrolyze
1.3.3.1.3. loss of a purine base from a nucleotide
1.3.3.2. deamination
1.3.3.2.1. rate of 100 bases per cell per day
1.3.3.2.2. cystine is deaminated into uracil
1.3.3.3. thymine dimers
1.3.3.3.1. UV radiation can cause covalent links to form between two adjacent pyrimidine bases
1.4. two pathways to remove dna damage
1.4.1. base excision repair
1.4.1.1. involves battery of DNA glycosylases (enzymes)
1.4.1.1.1. recognize a specific type of altered base in DNA
1.4.1.1.2. catalyze its hydrolytic removal
1.4.1.2. enzyme mediated flipping out of altered nucleotide from helix
1.4.1.2.1. find the damaged base
1.4.1.2.2. removes base from sugar
1.4.1.3. AP endonuclease
1.4.1.3.1. recognizes missing base
1.4.1.3.2. cuts the phosphodiester backbone and removes damage
1.4.1.3.3. resulting gap is repaired
1.4.1.3.4. depurinations directly repaired by AP endonucleases
1.4.2. nucleotide excision repair
1.4.2.1. can repair damage caused by almost any large change in the structure of the DNA double helix
1.4.2.1.1. bulky lesions
1.4.2.1.2. pyrimidine dimers
1.4.2.2. process
1.4.2.2.1. lrg multienzyme complex scans the DNA for a distortion in double helix
1.4.2.2.2. finds distortion
1.4.2.2.3. DNA helicase peels away a single strand oligonucleotide containing the lesion
1.4.2.2.4. large gap in DNA helix repaired by DNA polymerase and DNA ligase
1.4.3. both pathways
1.4.3.1. damage excised
1.4.3.2. original DNA sequence restored by DNA polymerase
1.4.3.2.1. uses undamaged strand as template
1.4.3.3. remaining break in double helix sealed by DNA ligase
1.4.4. different
1.4.4.1. way in which they remove damage from DNA
1.5. Double strand breaks
1.5.1. 2 distinct mechanisms
1.5.1.1. nonhomologous end joining
1.5.1.1.1. broken ends simply brought together and rejoined by DNA ligation
1.5.1.1.2. loss of one/more nucleotides at site of joining
1.5.1.1.3. common in mammalian somatic cells
1.5.1.2. homologous recombination
1.5.1.2.1. more accurate
1.5.1.2.2. occurs in newly replicated DNA
1.5.1.2.3. DNA repaired using sister chromatid as a template
1.5.2. most organisms employ both
1.5.2.1. nonhomologous predominates in humans
1.5.2.2. homologous used only during and shortly after DNA replication
1.5.2.2.1. S and G2 phases
1.5.2.2.2. sister chromatids available to serve as templates
1.6. strand directed mismatch repair system
1.6.1. proofreading system that removes replication errors by the polymerase
1.6.1.1. missed by proofreading exonuclease
1.6.2. detects potential for distortion in the DNA helix from the misfit between noncomplementary base pairs
1.6.3. prob
1.6.3.1. proofreading system could correct original stand by mistake
1.6.4. 2 proteins
1.6.4.1. MutS
1.6.4.1.1. binds specifically to mismatched base pair
1.6.4.2. MutL
1.6.4.2.1. scans nearby DNA for a nick
1.6.4.2.2. triggers degradation of the nicked strand
1.6.5. 3 step process
1.6.5.1. recognition of a mismatch
1.6.5.2. excision of the segment of DNA containing the mismatch
1.6.5.3. resynthesis of the excised segment using the old strand as a template
1.6.6. reduces errors made during replication by x100
2. initiation and completion of DNA replication in chromosomes
2.1. telomerase
2.1.1. end replication problem
2.1.1.1. synthesis of lagging strand occurs by use of okazakis
2.1.1.2. backstitching mechanism encounters a prob
2.1.1.2.1. replication fork reaches an end of a linear chromosome
2.1.1.2.2. no place to produce the RNA primer needed to start the last okazaki fragment
2.1.1.2.3. lagging strand gets shorter with each replication
2.1.1.3. solution
2.1.1.3.1. bacteria
2.1.1.3.2. eukaryotes
2.2. T-loop
2.2.1. found at the end of mammalian chromosomes
2.2.2. allows the cell to distinguish between the natural ends of chromosomes and the double strand DNA breaks
2.2.3. formed by protruding end looping back and tucking its single stranded terminus into the duplex DNA of the telomeric repeat sequence
2.2.4. provides the normal ends of chromosomes with a unique structure
2.2.4.1. protects from degradative enzymes
3. Proofreading mechanisms
3.1. DNA polymerase =self correcting
3.1.1. 1 mistake 4 every 10^9 nucleotides copied
3.1.2. mistake
3.1.2.1. mispairs
3.1.2.1.1. G-T
3.1.2.1.2. C-A
3.1.3. role of DNA polymerase
3.1.3.1. prevent wrong nucleotide being incorporated
3.1.3.2. prevent frequent mutations
3.2. 2 types
3.2.1. monitoring
3.2.1.1. DNA polymerase double checks the exact base pairing
3.2.2. exonucleolytic proofreading
3.2.2.1. error correcting rxn
3.2.2.2. DNA polymerase can correct the mismatched nucleotides
4. DNA replication mechanisms
4.1. sliding ring
4.1.1. holds a moving DNA polymerase onto the DNA
4.1.2. DNA polymerase has tendency to rapidly dissociate
4.1.2.1. recycled
4.1.2.2. difficult to form long DNA strands
4.1.3. sliding clamp
4.1.3.1. accessory protein
4.1.3.2. keeps polymerase firmly on DNA when it is moving
4.1.3.2.1. releases it when polymerase encounters a double stranded region of DNA
4.1.3.3. clamp protein forms a lrg ring around the DNA double helix
4.1.3.4. ring slides along the DNA as the polymerase moves
4.1.3.4.1. one side of the ring is bound to the back of the DNA polymerase
4.1.3.5. Clamp loader
4.1.3.5.1. hydrolyzes ATP as it loads the clamp on to a primer-template junction
4.1.3.5.2. leading strand
4.1.3.5.3. lagging strand
4.2. Replication machine
4.2.1. formed by the cooperation of proteins at a replication fork
4.2.2. lrg and orderly multienzyme complex
4.2.2.1. remains stationary with respect to surroundings
4.2.2.2. powered by nucleoside triphosphate hydrolyses
4.2.3. components
4.2.3.1. DNA helicase
4.2.3.1.1. opens the DNA helix
4.2.3.2. 2 DNA polymerases
4.2.3.2.1. work at the fork
4.2.3.2.2. 1 on leading strand
4.2.3.2.3. 1 on lagging strand
4.2.3.3. folded lagging strand
4.2.3.3.1. more efficient replication
4.2.3.3.2. facilitates loading of polymerase clamp each time an okazaki fragment is synthesized
4.2.3.3.3. series of unsealed okazaki fragments left behind
4.2.3.4. close association of proteins
4.2.3.4.1. linked together into a single unit
4.3. DNA topoisomerases
4.3.1. winding problem
4.3.1.1. every 10 bases replicated at the fork corresponds to one complete turn about the axis of the parental double helix
4.3.1.2. for a replication fork to move the entire chromosome ahead of the fork has to rotate rapidly
4.3.1.2.1. requires lots of energy for long chromosomes
4.3.2. proteins that form a swivel in the DNA helix
4.3.2.1. reversible nuclease
4.3.2.1.1. adds covalently to DNA backbone phosphate
4.3.2.1.2. reversible rxn
4.3.3. two types
4.3.3.1. topoisomerase 1
4.3.3.1.1. tyrosine in active site
4.3.3.1.2. produces a nick in the phosphodiester backbone
4.3.3.1.3. original phosphodiester bond energy is stored in the phosphotyrosine linkage
4.3.3.1.4. DNA replication can occur with the rotation of only a short length of helix
4.3.3.2. topoisomerase 2
4.3.3.2.1. forms a covalent linkage to both strands of the DNA helix at the same time
4.3.3.2.2. stimulated by sites on chromosomes where two helices cross over each other
4.3.3.2.3. steps
4.3.3.2.4. separates the interlocked DNA circles
4.4. DNA Topoisomerases
4.4.1. prevent DNA tangling During Replication
5. DNA replication
5.1. DNA structure
5.1.1. Nucleotides
5.1.1.1. comoponents
5.1.1.1.1. nitrogenous base
5.1.1.1.2. A 5-C sugar
5.1.1.1.3. one/more phosphate grps
5.1.1.2. covalently linked to form a backbone
5.1.1.3. =subunits of nucleic acids
5.1.2. double helix
5.1.2.1. 2 polynucleotide chains
5.1.2.2. held together by H-bonds between bases
5.1.2.3. complementary base pairing
5.1.2.3.1. A-T
5.1.2.3.2. G-C
5.1.2.4. chemical polarity
5.1.2.4.1. one end referred to as 3' and other end is 5'
5.1.2.4.2. two strands are antiparallel
5.1.2.5. very stable double helix
5.1.2.5.1. lrg H-bonds between bases
5.1.2.5.2. cannot be used as template unless it is opened
5.2. DNA replication models
5.2.1. dispersive
5.2.1.1. each generation of daughter DNA will contain a mixture of DNA from the parent strands and the newly synthesized DNA
5.2.2. semiconservative
5.2.2.1. each parent strand serves as a template for the synthesis of a new daughter strand
5.2.2.2. DNA acts as a template for its own duplication
5.2.2.2.1. complementary base pairing
5.2.2.3. cell copies/replicates genes b4 passing them on to its descendants
5.2.2.3.1. 8hrs in animal cell
5.2.2.3.2. will get no more than one/two bases wrong
5.2.3. conservative
5.2.3.1. the parent molecule remains intact after being copied
5.2.4. Meselson-Stahl experiment
5.2.4.1. observations supported the semiconservative model
5.2.5. incorrect models
5.2.5.1. both daughter strands would grow continuously
5.2.5.2. would require growth in both 5'-3' and the 3'5' directions
5.2.5.3. no enzyme that catalyzes 3'-5' nucleotide polymerization
5.3. DNA synthesis (bacteria)
5.3.1. Replication origins
5.3.1.1. where DNA synthesis begins
5.3.1.2. span approximately 100 nucleotide pairs
5.3.1.3. bacterial chromosomes have a single RO.
5.3.1.3.1. Eukaryotic chromosomes=multiple ROs
5.3.2. process begun by initiator proteins
5.3.3. Replication forks
5.3.3.1. localised replication region which moves along parental DNA helix
5.3.3.2. Y-shape
5.3.3.3. asymmetrical
5.3.3.3.1. 5'-3' direction=problem
5.3.3.3.2. one new strand made on template that runs in 3'-5' direction
5.3.3.3.3. other new strand made on template that runs in 5'-3' direction
5.3.4. DNA polymerase
5.3.4.1. DNA polymerase catalyses the addition to the 3' end
5.3.4.1.1. reversible rxn
5.3.4.1.2. new subunits added only to 3' end
5.3.4.2. DNA chain synthesis only in 5'-3' direction
5.3.4.2.1. only 5'-3' allows efficient error correction
5.3.4.2.2. 3'-5' polymerisation blocks further chain elongation
5.3.4.3. energy provided the incoming nucleotide
5.3.4.4. only one type in the cell
5.3.5. Opening up the double helix
5.3.5.1. DNA double helix must be opened up ahead of the replication fork
5.3.5.1.1. incoming deoxyribonucleoside triphosphates can form base pairs with the template strand
5.3.5.2. 2 types of replication proteins
5.3.5.2.1. DNA helicase
5.3.5.2.2. SSB proteins
5.4. How a DNA strand grows in the 5'-3' direction
5.4.1. backstitching manoeuvre
5.4.1.1. incorrect 3'-5' direction made discontinuously
5.4.1.2. DNA polymerase moves backward
5.4.1.3. new small pieces made in correct direction
5.4.1.4. 2 types of strands
5.4.1.4.1. lagging strand
5.4.1.4.2. leading strand